ORNL germanium detectors, employed at SURF, help physicists unlock the mysteries of the universe
This Oak Ridge National Laboratory (ORNL) article, highlights the MAJORANA DEMONSTRATOR at SURF.
This article was originally published by ORNL and is republished here with permission. See the original article here.
Ever since physicist Ernest Rutherford discovered the atomic nucleus in 1911, studying its structure and behavior has remained a challenging task. More than a century later, even with today’s high-tech tools for researching nuclear physics, mysteries of the universe abound.
Relying on leading-edge germanium detectors developed by researchers at the Department of Energy’s Oak Ridge National Laboratory, the scientific community pursues elusive nuclear processes to unlock persistent mysteries. Answers to questions they hope to resolve hold the potential to redefine the universe itself. Why does the universe contain more matter than antimatter? Can a particle be both a matter and antimatter version of itself? Is there a mismatch between what the Big Bang produced and what the Standard Model of Particle Physics suggests?
Long at the vanguard of international efforts to answer questions like these, ORNL’s contributions remain strong today. David

ORNL’s David Radford displays a germanium detector of his design (its central contact visible at the 3 in. mark), vital to the LEGEND research efforts.
Credit: Carlos Jones/ORNL, U.S. Dept. of Energy
Radford, head of the lab’s Fundamental Nuclear and Particle Physics section, is an internationally renowned expert in the field who has had an indelible impact on the development of germanium detectors. Vital experimentation tools at the forefront of fundamental physics research, germanium detectors are large, single crystals of germanium — a metallic element — used to detect radiation and enable incredibly precise energy measurements.
Radford currently leads the DOE-funded portion of the Large Enriched Germanium Experiment for Neutrinoless Double Beta Decay, known as the LEGEND-1000 DOE project, or LEGEND, with ORNL as its lead lab. LEGEND is a multi-national, multi-institutional project that relies on germanium detectors to reveal more about the elusive nature of neutrinos. A better understanding of neutrinos — the most abundant, mass-containing particles that we know of in the universe — could help unlock physics’ greatest mysteries.
With a collaboration comprising more than 250 researchers from around 50 international institutions, LEGEND’s global physics experiment will rely on a one-ton germanium detector array, shielded from background radioactivity in an underground laboratory. The project also involves funding from the National Science Foundation and multiple European agencies.
Throughout the history of the pursuit of neutrinoless double beta decay, “ORNL was the lead lab for a predecessor experiment, the Majorana Demonstrator at the Sanford Underground Research Facility in South Dakota. We were also in friendly competition, working hand-in-hand with the European GERDA experiment,” said Radford. “We contribute a lot to our European and U.S. colleagues on design and analysis codes.”
For decades, Radford has been a key contributor to many impactful, international germanium-detector projects, including Majorana, GRETA and GRETINA. To advance capabilities for the study of nuclear structure and nuclear astrophysics, he has made major contributions to germanium detector design and use as these complex scientific sentinels have grown in both size and effectiveness over decades.
“After both GERDA and Majorana were successful, we merged the collaborations — the European and the U.S. experiments — and formed the LEGEND collaboration. ORNL contributed the detector design that’s being used for LEGEND,” said Radford.
Germanium detector history
Since the 1960s, one constant in this ongoing pursuit of elusive subatomic knowledge has been the chemical element germanium. Situated in the carbon column of the periodic table between silicon and tin, germanium has properties of both metals and nonmetals. Dmitri Mendeleev predicted its presence in 1869, and Clemens Winkler identified it in a mineral sample in 1886, naming it after his nationality. However, half a century passed before many of its potential uses were discovered and exploited.
During WWII, germanium was primarily used in point-contact diodes for radar pulse detection. After the war, from the 1950s to the early 1970s, germanium’s development as a semiconductor in transistors paved the way for its use in solid-state electronics, until it was replaced by its more plentiful, less expensive neighbor in the periodic table, silicon. Subsequently, germanium has also been used in fiber optics, solar panels, night vision goggles, polymerization catalysts for plastics, and the infrared optics of airport security thermal cameras and scanners.
However, germanium detectors, first grown from and built around a single crystal of high-purity germanium, did not arrive until the mid-1960s. These groundbreaking tools ushered in a new investigative technique for nuclear physicists: high-resolution gamma-ray spectroscopy.
This process determines the structure of nuclei or isotopic composition of materials by analyzing gamma-ray spectra — a quest in search of the unique gamma-ray fingerprints that atomic nuclei possess. Germanium proved ideal for use in gamma-ray spectroscopy because of its effective balance between high detection efficiency and high energy resolution.
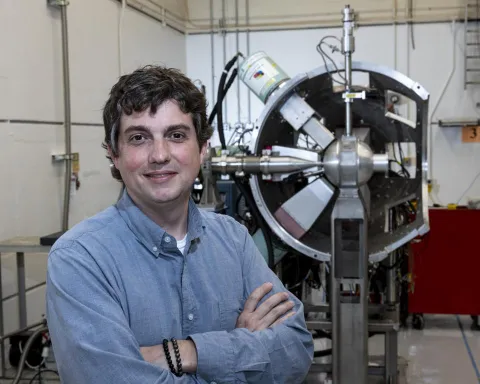
ORNL’s Mitch Allmond develops models, builds radiation detector systems and conducts experiments to explore the structure of atomic nuclei.
Credit: Carlos Jones/ORNL, U.S. Dept. of Energy
When a nucleus decays from an excited state into a lower-energy state, it usually does so by emitting a gamma ray — the highest-energy form of electromagnetic radiation — which, under the right conditions, is observable and characteristic of the initial and final quantum states. Herein lies the quest.
James “Mitch” Allmond, a research scientist at ORNL, studies low-energy nuclear physics and nuclear astrophysics, hoping to better understand the behaviors that emerge in nuclei as they move further from the “valley of stability,” where stable, long-lived nuclei reside. At DOE’s newest user facility, the Facility for Rare Isotope Beams, or FRIB, at Michigan State University, Allmond manages the ongoing FRIB Decay Station initiator project. He is particularly interested in how nuclear structure — the organization of protons and neutrons within an atomic nucleus — evolves for exotic radioactive isotopes. As these nuclei are produced farther from stability, new phenomena are being observed.
Normally, these rare isotopes only exist in stars or during events such as star mergers or supernovae, all of which lead to nucleosynthesis — the process in which lighter nuclei are used as building blocks to create new, often heavier atomic nuclei. Inside the resulting stars, nucleosynthesis has reoccurred, forming heavier elements through various fusion processes. With the help of germanium detectors, Allmond can study these rare isotopes, postulate theories about what is happening in the stars, and better understand the Earth’s elemental abundance.
“We’re really trying to understand the structure of atomic nuclei, which are quantum systems,” said Allmond, who is both an experimentalist and a theorist. “They can be excited, and they can decay. They often decay by gamma rays and often by more than one. We have ultimately been trying to measure multiple gamma rays emitted simultaneously by atomic nuclei to better understand nuclear structure and how that also impacts astrophysics and nucleosynthesis.”
Over decades, scientists have developed more advanced germanium detector arrays. In the 1990s, Radford dramatically advanced the point-contact design by increasing the contact area into what he called the “inverted coaxial” approach, allowing for a far larger germanium detector, exponentially increasing opportunities to view target phenomena.
“If the neutrino is a so-called Majorana particle, it is its own antineutrino,” said Radford. “That means the antineutrino and the neutrino are actually the same particle and that neutrinoless double beta decay should happen. That’s what we’re looking for. If we see it, we then know that lepton number [the difference between the number of leptons and the number of antileptons, which helps classify particles and interactions] is not conserved. That gives us a possible way to explain the matter/antimatter imbalance that the Big Bang created. It would change dramatically the Standard Model of Particle Physics — and perhaps even explain how we exist.”
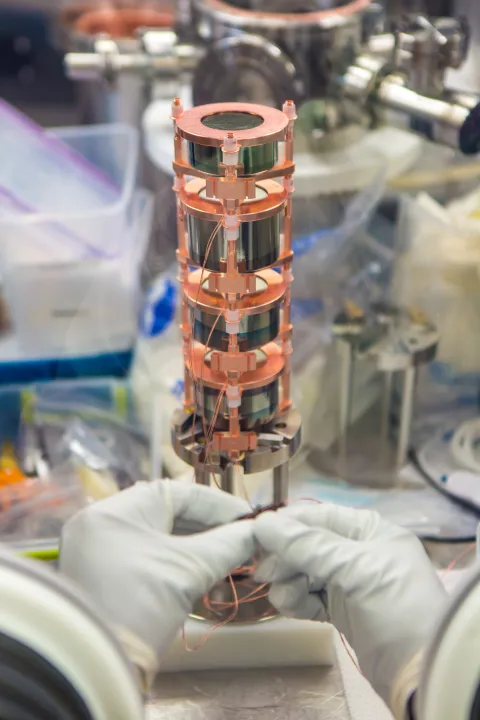
A researcher assembles a string of germanium detectors in a glovebox; multiple strings make up an array at the Majorana Demonstrator, a precursor experiment to LEGEND.
Credit: Matthew Kapust/Sanford Underground Research Facility
Chasing breakthroughs in low-energy nuclear structural science and neutrinoless double beta decay alike, leading researchers such as Radford and Allmond expect to continue to rely on germanium detectors for the foreseeable future — at ORNL and elsewhere.
Meanwhile, as nearly a dozen international experiments pursue the elusive Majorana neutrino, other detector technologies have emerged. Competing approaches use isotopes of xenon, molybdenum and tellurium. However, Radford maintains that germanium remains the preferred choice for major collaborations such as LEGEND. As Radford sees it, this collaborative, ongoing pursuit of the persistently elusive neutrinoless double beta decay — an effort to advance fundamental scientific knowledge of the universe — is foundational to our existence. He equates the quest for this knowledge with the paintings his wife Kathy, a fine artist, creates.
“Art is a cultural activity; humanity needs art,” Radford said. “Humanity should see the beauty around us, see things that challenge us and make us think and wonder. The science that we do, I think, is the same. We are curious. We, as humans, want to understand the world around us, to understand how we got to be here and how the world works. It’s one of the things we strive for. I’m very privileged to play some part in that goal…to understand how we’re here, how the universe works.”
The DOE Office of Science supported this research.
UT-Battelle manages ORNL for the Department of Energy’s Office of Science, the single largest supporter of basic research in the physical sciences in the United States. The Office of Science is working to address some of the most pressing challenges of our time. For more information, please visit energy.gov/science. — Chris Driver